What factors affect hemoglobin's oxygen affinity?
The oxyhemoglobin dissociation curve describes the relationship between arterial oxygen tension (partial pressure of oxygen in the arteries, PaO2) and the amount of oxygen bound to hemoglobin—the hemoglobin saturation. As arterial oxygen tension increases, the amount of oxygen loaded onto hemoglobin increases curvilinearly, creating a sigmoid- shaped graph—the result of enhanced oxygen-binding after the initial binding of oxygen occurs.
The upper portion of the curve (PaO2 > 60 mmHg) is flat (A), indicating that further increases in arterial oxygen tension do not result in significant increases in hemoglobin saturation. The lower and middle portions of the curve are steep (B), indicating major changes in oxyhemoglobin concentration with small changes in arterial oxygen tension.
P50 of hemoglobin
A useful parameter to describe the overall positioning of the curve is the P50—the arterial oxygen tension at which hemoglobin is 50% saturated. Normal P50, measured at 37°C and an arterial pH of 7.40, is 26.6 mmHg.
As hemoglobin’s affinity for oxygen decreases, oxygen is more readily unloaded at the tissue level. This is reflected in a rightward shift of the curve and a higher P50. A decrease in P50 indicates greater hemoglobin avidity for oxygen and decreased oxygen release.
Physiological factors that can shift the oxyhemoglobin
Changes in pH and the Bohr effect
Changes in the position of the curve with changes in red blood cell (RBC) intracellular hydrogen ion concentration constitute the Bohr effect. Decreases in pH shift the curve to the right, while increases shift the curve to the left.
Carbon dioxide
Carbon dioxide increases hydrogen ion concentration and lowers tissue pH. As a consequence, hemoglobin’s affinity for oxygen decreases and oxygen release to tissues is facilitated. Opposite changes occur in the lung.
Organophosphates
During glycolysis, red blood cells generate organophosphates, particularly 2,3-diphosphoglycerate (2,3-DPG). In red cells, due to the absence of mitochondria, 2,3-diphosphoglycerate is used for energy generation. In the setting of diminished oxygen availability (e.g., anemia, blood loss, chronic lung disease, high altitude, or right-to-left shunts), organophosphate production in red cells is increased, shifting the oxyhemoglobin curve to the right, thereby facilitating unloading of oxygen in peripheral tissues.
Changes in temperature
Hyperthermia shifts the curve to the right. Opposite changes occur with hypothermia.
Carbon monoxide levels
Carbon monoxide shifts the oxyhemoglobin dissociation curve to the left, impeding oxygen unloading in peripheral tissues. This effect is in addition to the effect of carbon monoxide in binding to hemoglobin and preventing oxygen loading in the lungs.
Methemoglobin
Methemoglobin is the result of oxidation of the iron moiety of hemoglobin from the ferrous to the ferric state. Intracellular enzymatic reductive pathways normally maintain methemoglobin levels of less than three percent.
In the presence of congenital deficiencies of reductive enzymes, or in the presence of oxidant drugs (e.g., antimalarials, dapsone, local anesthetics), methemoglobinemia may develop.
Methemoglobin shifts the oxyhemoglobin curve to the left, impairing oxygen release in peripheral tissues.
Presence of abnormal hemoglobins
Finally, the presence of abnormal hemoglobins—such as fetal hemoglobin in an adult—can have an effect on the oxygen-hemoglobin binding curve. Fetal hemoglobin, hemoglobin F, consists of two gamma chains replacing the normal two beta chains.
The oxyhemoglobin curve is shifted to the left in the presence of hemoglobin F, enhancing hemoglobin’s affinity for oxygen, an advantage during fetal life when arterial oxygen tension is low.
Become a great clinician with our video courses and workshops
Recommended reading
- Grippi, MA. 1995. “Gas exchange in the lung”. In: Lippincott's Pathophysiology Series: Pulmonary Pathophysiology. 1st edition. Philadelphia: Lippincott Williams & Wilkins. (Grippi 1995, 137–149)
- Grippi, MA. 1995. “Clinical presentations: gas exchange and transport”. In: Lippincott's Pathophysiology Series: Pulmonary Pathophysiology. 1st edition. Philadelphia: Lippincott Williams & Wilkins. (Grippi 1995, 171–176)
- Grippi, MA and Tino, G. 2015. “Pulmonary function testing”. In: Fishman's Pulmonary Diseases and Disorders, edited by MA, Grippi (editor-in-chief), JA, Elias, JA, Fishman, RM, Kotloff, AI, Pack, RM, Senior (editors). 5th edition. New York: McGraw-Hill Education. (Grippi and Tino 2015, 502–536)
- Tino, G and Grippi, MA. 1995. “Gas transport to and from peripheral tissues”. In: Lippincott's Pathophysiology Series: Pulmonary Pathophysiology. 1st edition. Philadelphia: Lippincott Williams & Wilkins. (Tino and Grippi 1995, 151–170)
- Wagner, PD. 2015. The physiologic basis of pulmonary gas exchange: implications for clinical interpretation of arterial blood gases. Eur Respir J. 45: 227–243. PMID: 25323225
Become an expert
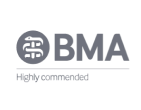
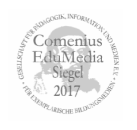